Gamma Rays and Natural Radiation
Nuclear logging instruments can be divided into passive and active instruments. Passive instruments contain only radiation detectors, and active instruments contain both a radioactive source and detectors.
- Passive tools measure natural radioactivity, usually by recording the gamma rays that are emitted by elements in the formation.
- Active tools measure induced radioactivity, which requires a radioactive source to emit neutrons or gamma rays that penetrate the borehole and the surrounding formation.
In this course, we will deal exclusively with the radioactivity naturally emitted from the formation. Density and neutron porosity logging tools (using induced radioactivity) are reviewed in other courses within this IPIMS topic.
Natural Radioactive Elements
Radioactivity is associated with the structure of chemical elements. An element contains protons and neutrons in its nucleus, along with electrons in one or more orbits. Each element is identified by its unique number of protons (Z-number). The majority of elements consist of a mixture of two, or more, isotopes.
Element isotopes have the same number of protons, but a different number of neutrons. Many isotopes are unstable and emit alpha, beta and/or gamma radiation, eventually decaying to a stable isotope. Of these three types of radiation, only the gamma rays can be recorded with logging tools in the wellbore because the beta and alpha particles have a very limited depth of penetration (often less than one cm in heavy materials). Gamma rays (photons) have a considerable penetration depth and thus allow the recording of natural gamma-radiation emitted by rocks through steel casing. Natural gamma ray logs can therefore be effectively acquired in both cased and open holes.
Origin of Natural Gamma Ray Radiation
The gamma rays encountered in the borehole can generally be attributed to three main sources in nature: the radioactive elements in the uranium group, the thorium group, and potassium:
- U-Ra: uranium-radium elements and their unstable daughter series of elements
- Th: thorium series
- K40: potassium-40 isotope
Uranium 235, uranium 238, and thorium 232 all decay to stable lead isotopes, by means of a long chain of daughter products. An isotope of potassium, K40, decays to argon, and gives off a gamma ray in the process.
Each type of decay is characterized by a gamma ray of a specific energy (wavelength), and the frequency of occurrence for each decay energy is different. Potassium-40 emits gamma rays with one single radiation energy (1.46 meV), whereas the U-Ra and Th series display a wide range of energies (Figure 1).
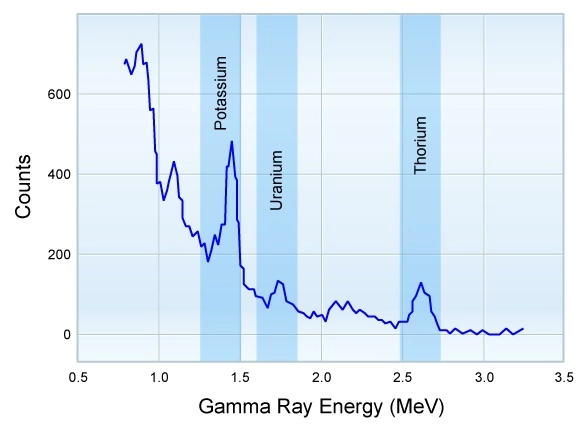
Figure 2 shows the relationship between gamma ray energy and the probability of emission per disintegration. This is an important concept because it is used as the basis for measurement in the natural gamma spectroscopy tools.
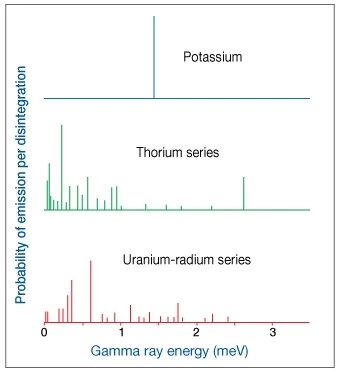
Radioactivity in Shales
The basic constituents of igneous rocks are (Figure 3):
- Quartz, with a low degree of radioactivity
- Feldspars, with variable radioactivity due to the different types of feldspar. Compositions of major elements in common feldspars can be expressed in terms of three end members: potassium feldspar end member KAlSi3O8 , albite end member NaAlSi3O8, anorthite end member CaAl2Si2O8. K40 is prevalent in the potassium feldspars.
- Micas, with K40 and sometimes U-Ra and Th
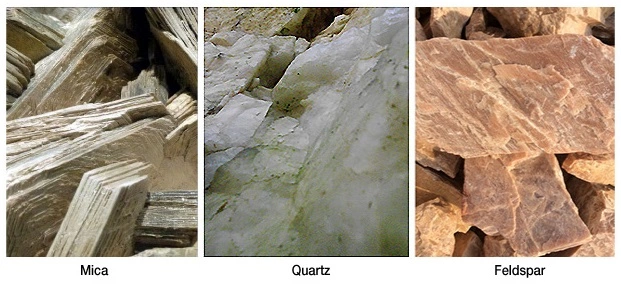
Feldspars decompose at a relatively rapid rate into clay minerals, resulting in the radioactive elements being trapped in the rock fabric. Because clay minerals are the principle constituents of shales, these are generally radioactive as well. A typical shale (Figure 4) might contain 6 ppm uranium, 12 ppm thorium, and 2% potassium. Because the various gamma ray sources are not all equally effective, it is more informative to consider this mix of radioactive materials on a common basis; for example, by reference to potassium equivalents (that is, the amount of potassium that produces the same number of gamma rays per unit of time).
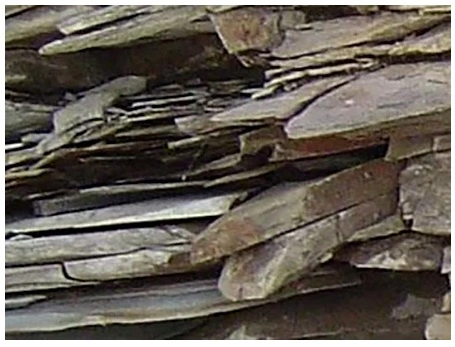
Shale is a mixture of clay minerals, sand, silts, and other extraneous materials and exhibits no “standard” gamma ray activity. Indeed, the main clay minerals vary enormously in their natural radioactivity (Figure 5): kaolinite has no potassium; illite has between 4% and 8% potassium, while montmorillonite less than 1%.
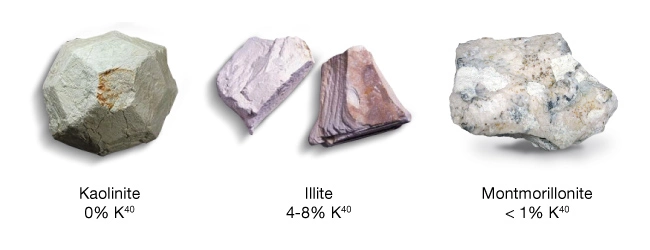
Occasionally, natural radioactivity may be due to the presence of dissolved potassium or other salts in shale pore water. Additionally, shale is not always the strongest gamma ray emitting lithology in a reservoir. Very good reservoir sandstones, found in some parts of the North Sea, contain large amounts of mica, which holds a significant amount of radioactive potassium. This situation can lead to gamma radiation levels in clean sandstones that are as high as levels in the surrounding shales.
The optimal solution to this interpretation challenge is to acquire spectral gamma ray log data. Consequently, a separate measurement of the potassium content will be made, enabling the mica’s radioactivity contribution to be separated from the shale radioactivity contribution, resulting in a more accurate shale volume and, subsequently, a more accurate porosity determination.
Gamma Ray Detectors
Scintillation counters have been used for many years to measure the radioactivity in boreholes. These counters are based on the physical phenomenon that when gamma rays interact with the lattice of the scintillation crystal, visible light flashes are produced. The most widely used crystal material has been the thallium-activated sodium iodide NaI, but other materials such as BGO (Bithmuth-germanium-oxide) and GSO (gadolinium oxy-ortho-silicate) have gained in popularity, due to their higher density and, therefore, more efficient conversion of gamma rays to scintillations. Figure 6 shows a schematic of a NaI detector. Some of the older generation of LWD natural gamma ray tools rely on several banks of Geiger-Muller tubes working together in order to accomplish their task.
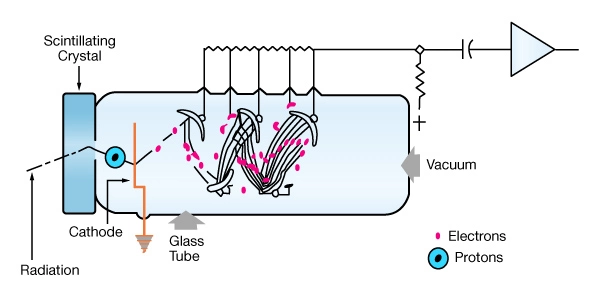
Operating Principle of Gamma Ray Logging Tools
When a gamma ray strikes the crystal, a single photon of light is emitted. This tiny flash of light then strikes a photo-cathode made from cesium-antimony or silver-magnesium. When the light quanta hit the photo-cathode of a photo-multiplier, they dislodge one or more electrons. These electrons are in turn accelerated by a cascade circuit of electrodes, where each subsequent electrode has a higher voltage, and more electrons are dislodged at each stage. This multiplication process leads to an avalanche of electrons that produce a measurable electric pulse at the last anode of the tube. An attractive feature of this technique is that the pulse height is proportional to the energy of the original gamma-photon allowing the detector to be used as a gamma ray spectroscopy device. The system has a very short unresponsive time and can register many counts per second without becoming swamped by numerous signals.